Hypothyroidism during the developmental stage induces disruption of hippocampal neurogenesis in later life, as well as inducing oxidative stress in the brain. The present study investigated the preventive effect of co-exposure to an antioxidant on disruptive neurogenesis induced by developmental exposure to anti-thyroid agent in rats. For this purpose, we used two antioxidants, α-glycosyl isoquercitrin (AGIQ) and α-lipoic acid (ALA). Mated female Sprague Dawley rats were either untreated (control) or treated with 12 ppm 6-propyl-2-thiouracil (PTU), an anti-thyroid agent, in drinking water from gestational day 6 to postnatal day (PND) 21, the latter group being subjected to feeding basal diet alone or diet containing AGIQ at 5,000 ppm or ALA at 2,000 ppm during PTU exposure. On PND 21, PTU-exposed offspring showed reductions in a broad range of granule cell lineage subpopulations and a change in the number of GABAergic interneuron subpopulations. Co-exposure of AGIQ or ALA with PTU altered the transcript levels of many genes across multiple functions, suggestive of enhancement of synaptic plasticity and neurogenesis. Nevertheless, immunohistochemical results did not support these changes. PTU exposure and co-exposure of AGIQ or ALA with PTU did not alter the hippocampal lipid peroxidation level. The obtained results suggest a possibility that thyroid hormone depletion itself primarily disrupts neurogenesis and that oxidative stress may not be involved in the disruption during development. Transcript expression changes of many genes caused by antioxidants may be the result of neuroprotective actions of antioxidants rather than their antioxidant activity. However, no preventive effect on neurogenesis suggested impairment of protein synthesis via an effect on mRNA translation due to hypothyroidism.
Introduction
The hippocampus is an important temporal lobe brain structure involved in cognition, learning, and memory. The hippocampus postnatally generates new neurons within the subgranular zone (SGZ) of the dentate gyrus (DG), which is termed “adult neurogenesis” (Fig. 1)1, 2. This neuronal production consists of multistep processes, including a number of developmental phases, such as self-renewal of neural stem cells, the facilitation of continued division of precursor cells to produce new granule cells, and subsequent differentiation and migration of these new cells into the granule cell layer (GCL)1, 2. In the hilus of the DG, subpopulations of γ-aminobutyric acid-ergic (GABAergic) interneurons innervate granule cell lineage populations to control neurogenesis in the SGZ2, 3. In addition to GABAergic neuronal inputs, various types of neurons outside the SGZ also create a synaptic connection with neurons in the DG, such as glutamatergic neurons in the entorhinal cortex providing axonal projections to the DG4 and cholinergic neurons originating from the septal nucleus and nucleus of the diagonal band of Broca innervating neurons in the hilus of DG4. Glutamatergic inputs to the SGZ are important for maintaining proper proliferation and differentiation of the granule cell lineage subpopulations3.
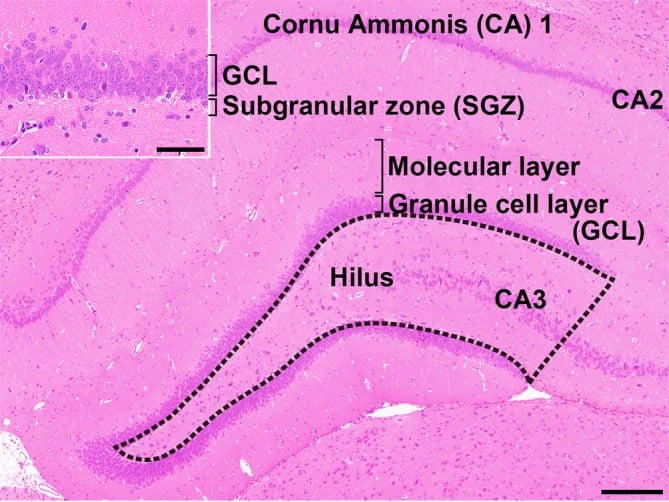
Fig. 1. Overview of the hippocampal formation of a male rat stained with hematoxylin and eosin. The number of cells in the hilus of the dentate gyrus (as enclosed by the dotted line) displaying immunoreactivity for parvalbumin (PVALB), reelin (RELN), calbindin-D-29K (CALB2), somatostatin (SST), or neuronal nuclei (NeuN) was counted and normalized for the unit area. Only small-sized cells with positive immunoreactivity for these antigens were counted, and larger Cornu Ammonis (CA) 3 neurons were excluded. (Inset) Higher magnification of the granule cell layer (GCL) and subgranular zone (SGZ). The numbers of immunoreactive cells for glial fibrillary acidic protein (GFAP); SRY box 2 (SOX2); T-box brain 2 (TBR2); doublecortin (DCX); NeuN; activity-regulated cytoskeleton-associated protein (ARC); Fos proto-oncogene, AP-1 transcription factor subunit (FOS); and cyclooxygenase 2 (COX2) and the number of proliferating cells or apoptotic cells were measured in the SGZ and/or GCL. Magnification ×40; bar 200 µm. (Inset) Higher magnification of the granule cell layer. Magnification ×400; bar 50 µm.
Thyroid hormones are crucial for brain development during fetal and neonatal periods. They play important roles in neuronal proliferation and migration, neuritogenesis, synaptogenesis, and myelinogenesis5. Previous studies have shown that rat developmental hypothyroidism causes aberrant brain growth involving diverse cellular populations and also impairs inherent brain structures and functions5. Hypothyroidism impairs neuronal migration and results in subcortical band heterotopia in the corpus callosum5, 6, as well as white matter hypoplasia with suppression of both axonal myelination and oligodendrocytic accumulation7. It has been considered that maternal hypothyroidism is associated with autism spectrum disorders (ASD)5. Multiple studies have highlighted the involvement of key processes, such as those including neurogenesis, neurite growth, synaptogenesis, and synaptic plasticity, in the pathophysiology of neurodevelopmental disorders, such as ASD8. Therefore, an experimental induction of developmental hypothyroidism could provide a reasonable model for ASD9.
Oxidative stress is defined as an imbalance between reactive oxygen species (ROS) production and the antioxidant defense system in an organism and is involved in various disorders such as neurodegenerative diseases and malignant tumors. Neural injury in the central and peripheral nervous system caused by some kinds of neurotoxicants is considered to be related to the induction of oxidative stress, but it is unclear how neurotoxicants cause oxidative stress and neurotoxicity. Importantly, SGZ cells in the DG generate ROS, because these cells have a high cellular activity for proliferation and differentiation requiring high oxygen demand10. With regard to the effect of hypothyroidism on brain tissues, induction of oxidative stress has been reported in the rat hippocampus as a result of administration of an anti-thyroid agent during the developmental stage or adult stage11, 12. Furthermore, co-exposure of extracts of a medicinal plant, Nigella sativa, with an anti-thyroid agent, 6-propyl-2-thiouracil (PTU), from the gestation period to adult stage in rats reduces apoptosis in the hippocampal DG, the Cornu Ammonis region (CA) 1 and CA3 areas, as compared with PTU exposure alone13. Therefore, there is a possibility that developmental exposure to an antioxidant may prevent hypothyroidism-related disruption of hippocampal neurogenesis induced by administration with an anti-thyroid agent during development in rats.
The present study was performed to clarify whether developmental exposure to an antioxidant has a potential to prevent hypothyroidism-related disruption of hippocampal neurogenesis induced by administration with PTU during development in rats. For this purpose, we used two antioxidants, α-glycosyl isoquercitrin (AGIQ) and α-lipoic acid (ALA), in the present study. AGIQ, also known as enzymatically modified isoquercitrin, is a flavonol glycoside derived by enzymatic glycosylation of rutin. AGIQ is a mixture of quercetin glycoside, consisting of isoquercitrin and its α-glucosylated derivatives, with 1–10 or more of additional linear glucose moieties and has greater water solubility and bioavailability14. AGIQ has been reported to exert anti-oxidative15, anti-inflammatory16, anti-hypertensive17, anti-allergic18 and tumor suppressive15, 19, 20 properties. ALA, a natural compound that is chemically named 5-(1,2-dithiolan-3-yl)pentanoic acid and is also known as thioctic acid21, is another metabolic antioxidant. In addition to direct antioxidant activity, ALA and its endogenous counterpart dihydrolipoic acid (DHLA), which is rapidly formed after uptake into the body’s cells, contributes to the nonenzymatic regeneration of reduced glutathione, vitamin C, vitamin E, and coenzyme Q10 in vivo22. Moreover, DHLA stimulates glutathione synthesis by enhancing cellular cysteine uptake. As DHLA is a supplier of reducing equivalents for the regeneration of detoxification enzymes, it is capable of supporting repair of oxidative damage22. ALA has been reported to prevent or ameliorate several ailments such as cardiovascular diseases, diabetic complications including retinopathy and neuropathy, and hypertension because of its antioxidant properties23. It is known that once absorbed in the body, AGIQ and ALA can pass the blood-brain barrier and be distributed in the brain24, 25. We have recently found that continuous AGIQ exposure from the developmental stage can facilitate fear extinction learning associated with enhancement of synaptic plasticity at the adult stage in rats26. Experimentally, ALA has been shown to ameliorate brain oxidative injury induced by methionine and choline deficiency27. Therefore, if oxidative stress is involved in aberrant neurogenesis in the hippocampal DG induced by developmental hypothyroidism, there is a possibility that co-exposure to AGIQ or ALA would suppress aberrant neurogenesis in the hippocampal DG.
Materials and Methods
Chemicals and animals
PTU (purity >99%; CAS No. 51-52-5) was purchased from MilliporeSigma (St. Louis, MO, USA). AGIQ (purity >97%) was supplied by San-Ei Gen F.F.I. Inc. (Osaka, Japan). DL-ALA (purity ≥99%; CAS No. 1077-28-7) was purchased from Tokyo Chemical Industry Co., Ltd. (Tokyo, Japan). Fifty mated female Slc:SD rats were purchased from Japan SLC, Inc. (Hamamatsu, Japan) at gestational day (GD) 1, where GD 0 was the day of appearance of a vaginal plug. Mated female rats were individually housed in polycarbonate cages with paper bedding until postnatal day (PND) 21, where PND 0 was defined as the day of delivery. Animals were maintained in an air-conditioned animal room (temperature, 23 ± 2°C; relative humidity, 55 ± 15%) with a 12-h light/dark cycle. Mated female rats were allowed to access to powdered basal diet (CRF-1, Oriental Yeast Co., Ltd., Tokyo, Japan) and tap water ad libitum until the start of developmental exposure to PTU with or without exposure to AGIQ or ALA. Offspring were weaned on PND 21 and thereafter reared three to five animals per cage and provided with powdered basal diet (CRF-1) and tap water ad libitum.
Experimental design
Mated female rats were randomly divided into four groups by stratified randomization according to the body weight on GD 5; 14 rats were fed basal diet and tap water (untreated controls), 12 rats were fed basal diet and water containing 12 ppm PTU (PTU alone), 12 rats were fed diet containing 5,000 ppm AGIQ and water containing 12 ppm PTU (PTU + AGIQ) or 12 rats were fed diet containing 2,000 ppm ALA and water containing 12 ppm PTU (PTU + ALA) (Fig. 2). Animals were treated from GD 6 to day 21 post-delivery with PTU with or without AGIQ or ALA. Based on a previous study that showed apparent aberrations in neuronal development in the hippocampal structure in offspring28, the PTU dose was set at 12 ppm. The chosen dosages of AGIQ and ALA have both been shown to suppress the promotion of hepatic preneoplastic lesions in rats19, 20.
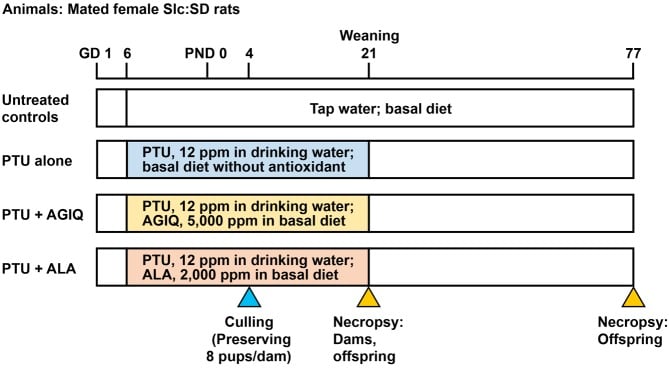
Fig. 2. Experimental design of maternal exposure to 6-propyl-2-thiouracil (PTU) from gestational day (GD) 6 to postnatal day (PND) 21 with or without co-exposure to α-glycosyl isoquercitrin (AGIQ) or α-lipoic acid (ALA). Dams in the untreated controls and PTU-exposed groups were euthanized on PND 21, and offspring in both the untreated controls and PTU-exposed groups were euthanized on PND 21 and PND 77.
Dams were subjected to measurement of body weight, and food and water consumption, twice a week between GD 6 and PND 21. On PND 4, the litters were randomly culled to preserve 6 or 7 male pups and 1 or 2 female pups per litter. If dams had fewer than 6 male pups, more female pups were included to maintain a total of 8 pups per litter. The offspring were weighed twice a week until PND 21. All dams and offspring were checked for general conditions in terms of appearance of abnormal gait and behaviors at the time of body weight measurement. Dams were euthanized by exsanguination from the abdominal aorta under CO2/O2 anesthesia on PND 21.
In the present study, male offspring were selected for immunohistochemical and gene expression analyses of the hippocampus because neurogenesis is influenced by circulating levels of steroid hormones during the estrous cycle29. On PND 21, 10 male offspring per group (1 pup per dam) were subjected to perfusion fixation for brain immunohistochemistry through the left cardiac ventricle with ice-cold 4% (w/v) paraformaldehyde (PFA) in 0.1 M phosphate buffer (pH 7.4) at a flow rate of 10 mL/min under deep anesthetization with CO2/O2. For transcript expression analysis, 6 male offspring per group (1 pup per dam) were euthanized by exsanguination from the abdominal aorta under CO2/O2 anesthesia and subjected to necropsy, and brains were removed and then fixed in methacarn solution at 4°C for 4 hours. For lipid peroxidation measurement, 6 to 8 male offspring per group (1 pup per dam) were euthanized by exsanguination from the abdominal aorta under CO2/O2 anesthesia and subjected to necropsy, and bilateral hippocampi were removed and stored at –80°C. All female offspring were similarly euthanized under anesthesia and subjected to necropsy, and brain tissues were removed and stored at –80°C. The remaining male offspring were maintained without exposure to PTU, AGIQ, or ALA until PND 77, and body weight, as well as food and water consumption, was measured once a week.
On PND 77, 8 to 10 male offspring per group (1 pup per dam) were subjected to perfusion fixation with ice-cold 4% PFA buffer solution for brain immunohistochemistry at a flow rate of 35 mL/min. For transcript expression analysis, 6 to 8 male offspring per group (1 pup per dam) were subjected to necropsy, and removed brains were fixed in methacarn solution.
The dosing schedule of PTU and necropsy time points of the present study were identical to those in a previous study6, following recommendations in the Organization for Economic Co-operation and Development (OECD) guideline for the testing of chemicals (Test No. 426: Developmental Neurotoxicity Study)30. All procedures in this study were conducted in accordance with the Guidelines for Proper Conduct of Animal Experiments (Science Council of Japan, 1 June 2006) and according to the protocol approved by the Animal Care and Use Committee of Tokyo University of Agriculture and Technology. All efforts were made to minimize animal suffering.
Immunohistochemistry and apoptotic cell detection
After perfusion with 4% PFA buffer solution on PND 21 and PND 77, brains were additionally immersed in the same solution overnight at 4°C. In untreated controls, 3-mm-thick coronal slices were prepared at −3.0 mm from the bregma on PND 21 and at −3.5 mm from the bregma on PND 77. In the PTU-exposure group, brain size was turned out to be small, and coronal slices were prepared at the proportionally similar position to the untreated controls. Brain slices were immersed in 4% PFA buffer solution overnight at 4°C and were routinely processed for paraffin embedding and sectioned into 3-μm-thick slices.
Brain sections from offspring on PND 21 and PND 77 were subjected to immunohistochemistry using primary antibodies against the following antigens: proliferating cell nuclear antigen (PCNA), a cell proliferation marker in the SGZ; glial fibrillary acidic protein (GFAP), which is expressed in type-1 neural stem cells (radial glial cells) in the SGZ and astrocytes2; SRY box 2 (SOX2), which is expressed in type-1 neural stem cells and type-2a progenitor cells in the SGZ1; T-box brain 2 (TBR2), which is expressed in type-2b progenitor cells in the SGZ1; doublecortin (DCX), which is expressed in type-2b and type-3 progenitor cells and immature granule cells in the SGZ and GCL2; neuronal nuclei (NeuN), which is expressed in postmitotic neurons of both immature and mature granule cells in the SGZ and GCL2; and reelin (RELN), parvalbumin (PVALB), calbindin-D-29K (CALB2), and somatostatin (SST), which are expressed in GABAergic interneurons in the DG hilar region3; activity-regulated cytoskeleton associated protein (ARC), Fos proto-oncogene, AP-1 transcription factor subunit (FOS), and cyclooxygenase 2 (COX2), which are members of the immediate-early genes involved in synaptic plasticity31, 32 in the GCL. The respective primary antibodies were applied to brain sections for incubation overnight at 4°C. The primary antibodies are listed in Supplementary Table 1 (online only). One section per animal was subjected to immunohistochemistry of each molecule.
To block endogenous peroxidase, deparaffinized sections were incubated in 0.3% (v/v) H2O2 solution in absolute methanol for 30 min. The antigen retrieval conditions that were applied for some antibodies are listed in Supplementary Table 1 (online only). Immunodetection was conducted using a Vectastain® Elite ABC kit (Vector Laboratories Inc., Burlingame, CA, USA) with 3,3’-diaminobenzidine (DAB)/H2O2 as the chromogen. Hematoxylin counterstaining was then performed, and coverslips were mounted on immunostained sections for microscopic examination.
To evaluate apoptosis in the SGZ of the DG in the offspring, a terminal deoxynucleotidyl transferase dUTP nick-end labeling (TUNEL) assay was performed using an ApopTag Peroxidase In Situ Apoptosis Detection Kit (MilliporeSigma) according to the manufacturer’s instructions, with DAB/H2O2 as the chromogen. One section per animal was subjected to a TUNEL assay.
Evaluation of immunoreactive cells and apoptotic cells
Immunoreactive cells, i.e., PCNA+, GFAP+, SOX2+, TBR2+, DCX+, NeuN+, ARC+, FOS+, and COX2+ cells or TUNEL+ apoptotic cells, in the SGZ and/or GCL were bilaterally counted and normalized for the length of the SGZ (Fig. 1). Immunoreactive cells distributed within the hilus of the hippocampal DG, i.e., RELN+, PVALB+, CALB2+, SST+, or NeuN+ cells, were bilaterally counted and normalized per area unit of the hilar area (Fig. 1).
Immunoreactive neurons located inside of the CA3, consisting of large pyramidal neurons that can be morphologically distinguished from relatively small interneurons, were excluded from counting immunoreactive cells in the hilus of the DG. The number of each immunoreactive cellular population (except for NeuN+ cells in the GCL) or TUNEL+ apoptotic cells was manually counted under microscopic observation using a BX53 microscope (Olympus Corporation, Tokyo, Japan). In the case of NeuN+ cells in the GCL, the number of immunoreactive cells for counting was high, and therefore, an image analysis-assisted automatic cell counting method was applied. More specifically, digital photomicrographs at ×200-fold magnification were taken using a DP72 Digital Camera System (Olympus Corporation) attached to a BX53 microscope, and positive cell counting was performed by applying the WinROOF image analysis software package (version 5.7; Mitani Corporation, Fukui, Japan). The length of the SGZ and the hilar area were measured in microscopic images at ×40-fold magnification by applying the cellSens Standard (version 1.9; Olympus Corporation).
Transcript expression analysis
Transcript expression levels in the hippocampal DG were examined using real-time reverse-transcription polymerase chain reaction in offspring on PND 21 and PND 77. Brain tissues were dissected according to the whole-brain fixation method using methacarn solution as previously reported33. In brief, 2-mm-thick coronal cerebral slices were prepared at the position of −3.0 mm from the bregma. Hippocampal DG tissues were collected from the slice using a punch-biopsy device with a pore size of 1 mm in diameter (Kai Industries Co., Ltd., Gifu, Japan). Total RNA was extracted from tissue samples from each group (n=6 per group at both PND 21 and PND 77) using an AllPrep DNA/RNA Mini Kit (Qiagen, Hilden, Germany). First-strand cDNA was synthesized using SuperScript® III Reverse Transcriptase (Thermo Fisher Scientific, Waltham, MA, USA) in a 20-μL total reaction mixture with 20 or 200 ng of total RNA. Analysis of the transcript levels for gene targets shown in Supplementary Table 2 (online only) was performed using PCR primers designed with the Primer Express software (Version 3.0; Thermo Fisher Scientific). Real-time PCR with Power SYBR® Green PCR Master Mix (Thermo Fisher Scientific) was conducted using a StepOnePlusTM Real-time PCR System (Thermo Fisher Scientific). The relative differences in gene expression between untreated controls and each treatment group were calculated using threshold cycle (CT) values that were first normalized to hypoxanthine phosphoribosyltransferase 1 (Hprt1) or glyceraldehyde-3-phosphate dehydrogenase (Gapdh), which served as endogenous controls in the same sample, and then relative to a control CT value using the 2−ΔΔCT method34.
Lipid peroxidation measurement
Thiobarbituric acid reactive substances (TBARS) levels, as an index of lipid peroxidation, in whole hippocampal tissue of male offspring on PND 21 were measured using a Malondialdehyde Assay Kit (Northwest Life Science Specialties, LLC, Vancouver, WA, USA), according to the manufacturer’s instructions (n=6 to 8 per group; 1 pup per dam). The amount of malondialdehyde (MDA) was determined spectrophotometrically at 532 nm. The measured values were expressed as nmol MDA/g organ weight.
Statistical analysis
Numerical data are presented as the mean ± SD. Maternal body weights and brain weights were analyzed using the individual animal as the experimental unit. Offspring body weights, brain weights, immunoreactive cell counts for each antigen and the number of apoptotic cells, and transcript expression data were analyzed using the litter as the experimental unit. Data were analyzed using Levene’s test for homogeneity of variance. If the variance was homogenous, numerical data were evaluated using Tukey’s multiple comparison tests to compare between untreated controls and each treatment group or between the PTU-alone group and each of PTU + antioxidant group. For heterogeneous data, Aspin-Welch’s t-test with Bonferroni correction was used. All analyses were performed using the IBM SPSS Statistics ver. 25 (IBM Corporation, Armonk, NY, USA), and P<0.05 was considered statistically significant.
Results
Maternal parameters
One nonpregnant animal in the PTU + AGIQ group was excluded from the experiment. Therefore, the effective numbers of dams were 14, 12, 11, and 12 for the untreated controls, PTU-alone, PTU + AGIQ, and PTU + ALA groups, respectively. The numbers of implantation sites and live offspring and male ratio were not different between the untreated controls and each PTU-exposed group and between the PTU-alone group and each antioxidant co-exposure group (Supplementary Table 3: online only). Dams showed significant decreases in body weight from PND 4 to PND 9 in the PTU-alone group and from GD 21 to PND 21 in the PTU + ALA group compared with the untreated controls (Supplementary Table 4: online only). Dams in the PTU + ALA group also showed a significant decrease in body weight from PND 4 to PND 21 compared with the PTU-alone group (Supplementary Table 4: online only). Food consumption was significantly decreased on GD 14 and GD 17 and from PND 1 to PND 20 in the PTU-alone group and PTU + AGIQ group and from GD 6 to PND 20 in the PTU + ALA group compared with the untreated controls. The PTU + ALA group showed significant decreases in food consumption on GD 10, on PND 6, and from PND 13 to PND 20 compared with the PTU-alone group (Supplementary Table 5: online only). Water consumption was significantly decreased from PND 1 to PND 20 in the PTU-alone group and PTU + AGIQ group and from GD 6 to GD 14 and PND 1 to PND 20 in the PTU + ALA group compared with the untreated controls. The PTU + ALA group also showed a significant decrease in water consumption on GD 10, GD 14, and PND 6 and from PND 13 to PND 20 compared with the PTU-alone group (Supplementary Table 6: online only). At necropsy on PND 21, the PTU + ALA group showed a significant decrease in body weight compared with the untreated controls and PTU-alone group (Supplementary Table 3: online only). Based on the mean values of water consumption, dams in each PTU-exposure group received 1.34, 1.37, and 1.18 mg/kg body weight/day PTU during the gestation period in the PTU-alone group, PTU + AGIQ group, and PTU + ALA group, respectively. The dams of each group received 2.29, 2.30, and 2.19 mg/kg body weight/day PTU during the lactation period, respectively. With regard to the consumption of antioxidants, dams in the antioxidant co-exposure groups received 274.7 mg/kg body weight/day AGIQ and 96.9 mg/kg body weight/day ALA during the gestation period, based on the mean values of food consumption. During the lactation period, dams in the antioxidant co-exposure groups received 544.5 mg/kg body weight/day AGIQ and 205.8 mg/kg body weight/day ALA.
Necropsy data of male offspring
Body weights of male offspring on PND 21 and PND 77 were significantly lower in the PTU-alone group, PTU + AGIQ group, and PTU + ALA group compared with the untreated controls (Supplementary Table 7: online only). Male offspring in the PTU + ALA group showed a significant decrease in body weight on PND 21 compared with the PTU-alone group. Brain weight in male offspring on PND 21 and PND 77 was significantly lower in the PTU-alone group, PTU + AGIQ group, and PTU + ALA group compared with the untreated controls.
Numbers of granule cell lineage subpopulations in the SGZ and GCL of male offspring
On PND 21, the numbers of GFAP+ cells and TBR2+ cells in the SGZ and NeuN+ cells in the SGZ and GCL were significantly less in the PTU-alone group compared with the untreated controls (Fig. 3, Supplementary Fig. 1: online only). With regard to the numbers of SOX2+ cells in the SGZ and DCX+ cells in the SGZ and GCL, there were no significant differences in the PTU-alone group compared with the untreated controls. The number of DCX+ cells was significantly decreased in the PTU + AGIQ group. There were no significant differences in the numbers of GFAP+ cells, SOX2+ cells, TBR2+ cells, DCX+ cells, and NeuN+ cells between any antioxidant co-exposure group and the PTU-alone group.
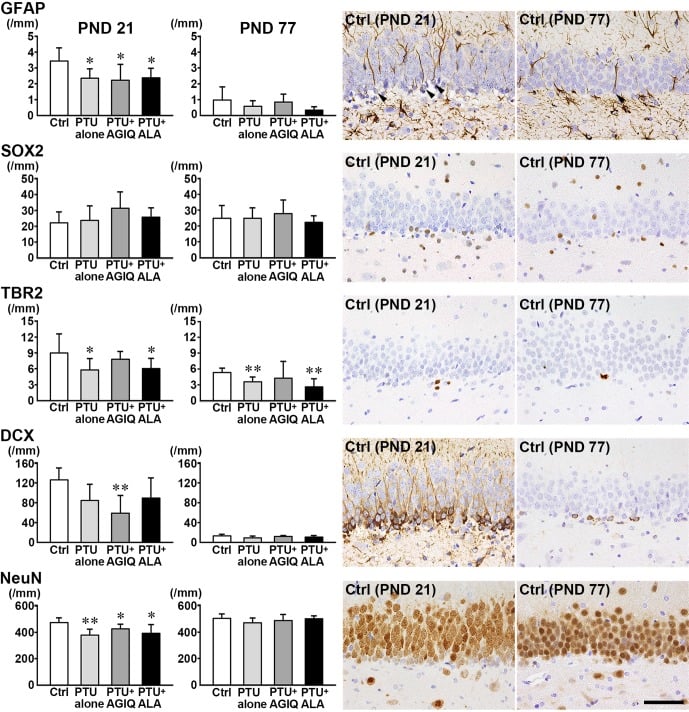
Fig. 3. The numbers of granule cell lineage subpopulations immunoreactive for glial fibrillary acidic protein (GFAP), SRY box 2 (SOX2), and T-box brain 2 (TBR2) in the subgranular zone (SGZ), and doublecortin (DCX), and neuronal nuclei (NeuN) in the SGZ and granule cell layer (GCL) of the dentate gyrus of male offspring on postnatal day (PND) 21 and PND 77. Graphs show the numbers of immunoreactive cells in the SGZ and/or GCL. Data are expressed as the mean + SD. N=10/group on PND 21, and N=8–10 on PND 77 (untreated controls, 10; 6-propyl-2-thiouracil (PTU)-alone group, 10; PTU + α-glycosyl isoquercitrin (AGIQ) group, 9; PTU + α-lipoic acid (ALA) group, 8). Representative images from the untreated controls on PND 21 (left) or on PND 77 (right). Arrowheads indicate immunoreactive cells. Magnification ×400; bar 50 µm. *Significantly different from untreated controls by Tukey’s test or Aspin-Welch’s t-test with Bonferroni correction (P<0.05). **Significantly different from untreated controls by Tukey’s test or Aspin-Welch’s t-test with Bonferroni correction (P<0.01).
On PND 77, the number of TBR2+ cells was significantly less in the PTU-alone group compared with the untreated controls (Fig. 3, Supplementary Fig. 2: online only). No significant differences were observed in the numbers of GFAP+ cells, SOX2+ cells, DCX+ cells, and NeuN+ cells in the PTU-alone group compared with the untreated controls. There were no significant differences in the number of GFAP+ cells, SOX2+ cells, TBR2+ cells, DCX+ cells, and NeuN+ cells between any antioxidant co-exposure group and the PTU-alone group.
Number of neuronal subpopulations in the DG hilar region of male offspring
On PND 21, the number of PVALB+ cells in the hilus was significantly less in the PTU-alone group compared with the untreated controls (Fig. 4, Supplementary Fig. 3: online only). There were no significant differences in the numbers of RELN+ cells, CALB2+ cells, SST+ cells, and NeuN+ postmitotic neurons in the PTU-alone group compared with the untreated controls. No significant differences were observed in the numbers of PVALB+ cells, RELN+ cells, CALB2+ cells, SST+ cells, and NeuN+ postmitotic neurons between any antioxidant co-exposure group and the PTU-alone group.
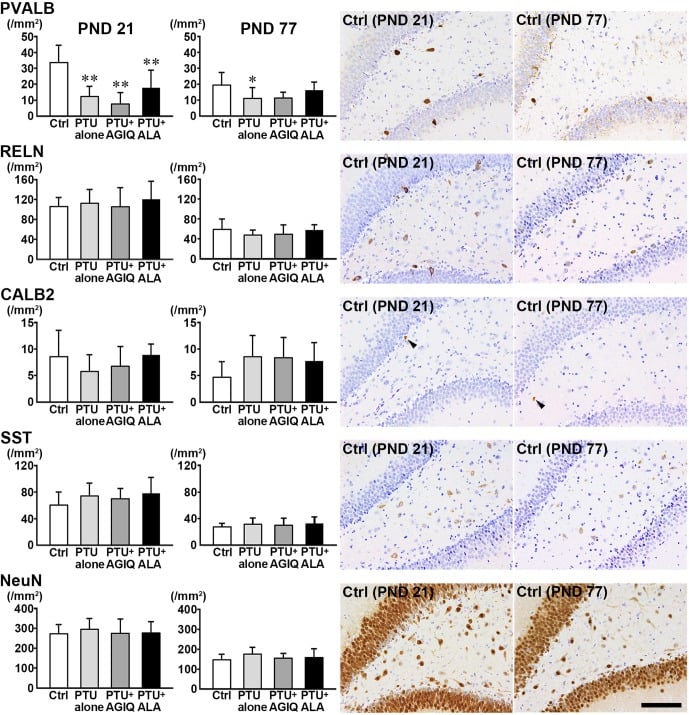
Fig. 4. The numbers of γ-aminobutyric acid-ergic (GABAergic) interneurons immunoreactive for parvalbumin (PVALB), reelin (RELN), calbindin-D-29K (CALB2) and somatostatin (SST) and those of neuronal nuclei (NeuN)-immunoreactive postmitotic neurons in the hilus of the dentate gyrus of male offspring on postnatal day (PND) 21 and PND 77. The graphs show the densities of immunoreactive cells in the hilus of the dentate gyrus. Data are expressed as the mean + SD. N=10/group on PND 21, and N=8–10 on PND 77 (untreated controls, 10; 6-propyl-2-thiouracil (PTU)-alone group, 10; PTU + α-glycosyl isoquercitrin (AGIQ) group, 9; PTU + α-lipoic acid (ALA) group, 8). Representative images from the untreated controls on PND 21 (left) or on PND 77 (right). Arrowheads indicate immunoreactive cells. Magnification ×200; bar 100 µm. *Significantly different from untreated controls by Tukey’s test or Aspin-Welch’s t-test with Bonferroni correction (P<0.05). **Significantly different from untreated controls by Tukey’s test or Aspin-Welch’s t-test with Bonferroni correction (P<0.01).
On PND 77, the number of PVALB+ cells was significantly less in the PTU-alone group compared with the untreated controls (Fig. 4, Supplementary Fig. 4: online only). There were no significant differences in the numbers of RELN+ cells, CALB2+ cells, SST+ cells, and NeuN+ postmitotic neurons in the PTU-alone group compared with the untreated controls. No significant differences were observed in the numbers of PVALB+ cells, RELN+ cells, CALB2+ cells, SST+ cells, and NeuN+ postmitotic neurons between any antioxidant co-exposure group and the PTU-alone group.
Proliferating and apoptotic cells in the SGZ and GCL of male offspring
On PND 21 and PND 77, there were no significant differences in the numbers of PCNA+ proliferating cells in the SGZ and TUNEL+ apoptotic cells in the SGZ and GCL in the PTU-alone group compared with the untreated controls (Fig. 5, Supplementary Fig. 5 and 6: online only). No significant differences were observed in the numbers of PCNA+ cells and TUNEL+ cells between any antioxidant co-exposure group and the PTU-alone group.
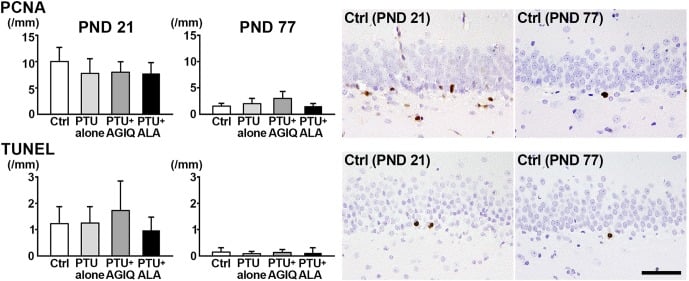
Fig. 5. The numbers of proliferating cell nuclear antigen (PCNA)+ cells in the subgranular zone (SGZ) and terminal deoxynucleotidyl transferase dUTP nick end labeling (TUNEL)+ cells in the SGZ and granule cell layer (GCL) of male offspring on postnatal day (PND) 21 and PND 77. The graphs show the numbers of positive cells in the SGZ and/or GCL. Data are expressed as the mean + SD. N=10/group on PND 21, and N=8–10 on PND 77 (untreated controls, 10; 6-propyl-2-thiouracil (PTU)-alone group, 10; PTU + α-glycosyl isoquercitrin (AGIQ) group, 9; PTU + α-lipoic acid (ALA) group, 8). Representative images from the untreated controls on PND 21 (left) or on PND 77 (right). Magnification ×400; bar 50 µm.
Numbers of immunoreactive cells for synaptic plasticity-related molecules in the GCL of male offspring
On PND 21, the numbers of ARC+ cells and FOS+ cells in the GCL were significantly less in the PTU-alone group compared with the untreated controls (Fig. 6, Supplementary Fig. 7: online only). The number of COX+ cells was significantly decreased in the PTU + AGIQ group. No significant differences were observed in the numbers of ARC+ cells, FOS+ cells, and COX2+ cells in the GCL between any antioxidant co-exposure group and the PTU-alone group.
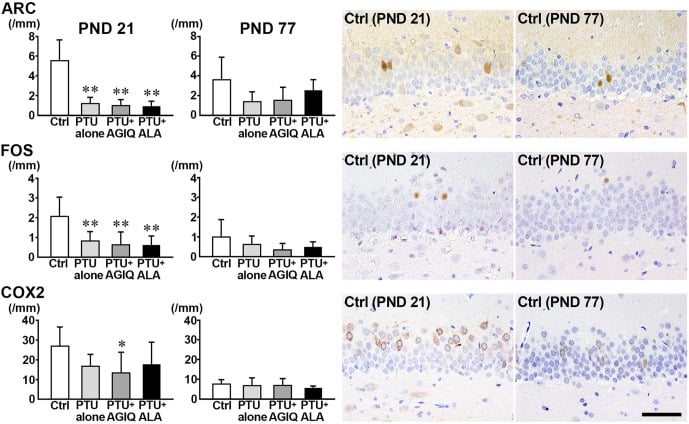
Fig. 6. The numbers of immunoreactive cells for activity-regulated cytoskeleton associated protein (ARC), Fos proto-oncogene, AP-1 transcription factor subunit (FOS), and cyclooxygenase 2 (COX2) in the granule cell layer (GCL) of male offspring on postnatal day (PND) 21 and PND 77. The graphs show the numbers of immunoreactive cells in the GCL. Data are expressed as the mean + SD. N=10/group on PND 21, and N=8–10 on PND 77 (untreated controls, 10; 6-propyl-2-thiouracil (PTU)-alone group, 10; PTU + α-glycosyl isoquercitrin (AGIQ) group, 9; PTU + α-lipoic acid (ALA) group, 8). Representative images from the untreated controls on PND 21 (left) or on PND 77 (right). Magnification ×400; bar 50 µm. *Significantly different from untreated controls by Tukey’s test or Aspin-Welch’s t-test with Bonferroni correction (P<0.05). **Significantly different from untreated controls by Tukey’s test or Aspin-Welch’s t-test with Bonferroni correction (P<0.01).
On PND 77, no significant differences were observed in the numbers of FOS+ cells, ARC+ cells, and COX2+ cells in the PTU-alone group compared with the untreated controls (Fig. 6, Supplementary Fig. 8: online only). No significant differences were observed in the numbers of FOS+ cells, ARC+ cells, and COX2+ cells between any antioxidant co-exposure group and the PTU-alone group.
Transcript expression changes in the DG of male offspring
On PND 21, no significant difference was observed in the transcript levels of granule cell lineage-related Sox2 and Dcx after normalization with Gapdh and Hprt1 between the PTU-alone group and untreated controls (Table 1). Eomes (also known as Tbr2) showed a significantly decreased transcript level after normalization with Hprt1 in the PTU-alone group compared with the untreated controls. The transcript level of Sox2 was not significantly changed after normalization with Gapdh and Hprt1 in the PTU + AGIQ group and PTU + ALA group compared with the PTU-alone group. Eomes significantly increased after normalization with Gapdh and Hprt1 in the PTU + ALA group compared with the PTU-alone group. The transcript level of Dcx significantly increased after normalization with Gapdh and Hprt1 in the PTU + AGIQ group and PTU + ALA group compared with the PTU-alone group. The transcript level of interneuron subpopulation-related Pvalb significantly decreased after normalization with Gapdh and Hprt1 in the PTU-alone group compared with the untreated controls. Regarding the transcript levels of Reln and Sst, no significant difference was observed after normalization with Gapdh and Hprt1 between the PTU-alone group and untreated controls. No significant difference was observed in the transcript level of Pvalb after normalization with Gapdh and Hprt1 between any antioxidant co-exposure group and the PTU-alone group. The transcript levels of Reln and Sst significantly increased after normalization with Gapdh and/or Hprt1 in the PTU + AGIQ group and PTU + ALA group compared with the PTU-alone group. With regard to glutamate receptor-related genes, no significant difference was observed in the transcript levels of Gria1 and Gria2 after normalization with Gapdh and Hprt1 between the PTU-alone group and untreated controls. The transcript level of Gria3, Grin2a, and Grin2b significantly decreased after normalization with Gapdh and Hprt1 in the PTU-alone group compared with the untreated controls. The transcript levels of Gria1, Gria2, Gria3, Grin2a, and Grin2b significantly increased after normalization with Gapdh and Hprt1 in the PTU + AGIQ group and PTU + ALA group compared with the PTU-alone group. With regard to cholinergic receptor Chrna7, no significant difference was observed in the transcript level after normalization with Gapdh and Hprt1 in the PTU-alone group compared with the untreated controls. The transcript level of Chrna7 significantly increased after normalization with Gapdh in the PTU + AGIQ group when compared with the PTU-alone group. With regard to neurotrophic factor/receptor-related genes, no significant differences were observed in the transcript levels of Bdnf and Ntrk2 after normalization with Gapdh and Hprt1 in the PTU-alone group compared with the untreated controls. The transcript level of Bdnf significantly increased after normalization with Gapdh and Hprt1 in the PTU + AGIQ group and PTU + ALA group compared with the PTU-alone group. With regard to synaptic plasticity-related genes, the transcript levels of Fos, Epha4, and Ephb2 significantly decreased after normalization with Gapdh and/or Hprt1 in the PTU-alone group compared with the untreated controls. There were no significant differences in the transcript levels of Arc, Efnb3, Ephb1, and Ptgs2 after normalization with Gapdh and Hprt1 in the PTU-alone group compared with the untreated controls. The transcript levels of Arc, Epha4, and Ptgs2 significantly increased after normalization with Gapdh and/or Hprt1 in the PTU + AGIQ group and PTU + ALA group compared with the PTU-alone group. The transcript level of Efnb3 significantly increased after normalization with Gapdh and Hprt1 in the PTU + AGIQ group compared with the PTU-alone group. The transcript level of Ephb1 significantly decreased after normalization with Gapdh and Hprt1 in the PTU + AGIQ group compared with the PTU-alone group. With regard to stem cell factor/receptor-related genes, no significant difference was observed in the transcript levels of Kitlg and Kit after normalization with Gapdh and Hprt1 in the PTU-alone group compared with the untreated controls. The transcript level of Kitlg significantly decreased after normalization with Gapdh and Hprt1 in the PTU + AGIQ group and PTU + ALA group compared with the PTU-alone group. The transcript level of Kit significantly increased after normalization with Gapdh and/or Hprt1 in the PTU + AGIQ group and PTU + ALA group compared with the PTU-alone group. With regard to growth factor receptor-related genes, no significant difference was observed in the transcript levels of Igf1r and Igf2r after normalization with Gapdh and Hprt1 in the PTU-alone group compared with the untreated controls. The transcript level of Igfr1 significantly increased after normalization with Gapdh and Hprt1 in the PTU + ALA group compared with the PTU-alone group. The transcript level of Ifg2r significantly increased after normalization with Gapdh in the PTU + AGIQ group and PTU + ALA group compared with the PTU-alone group. With regard to cell proliferation-related Pcna, the transcript level showed no significantly different change between the PTU-alone group and untreated controls or between any antioxidant co-exposure group and the PTU-alone group. With regard to anti-cell proliferation-related Cdkn1a, the transcript level significantly decreased after normalization with Gapdh in the PTU-alone group compared with the untreated controls. The transcript levels of Cdkn1a significantly decreased after normalization with Gapdh and Hprt1 in the PTU + AGIQ group and PTU + ALA group compared with the PTU-alone group. With regard to apoptosis-related genes, no significant differences were observed in the transcript levels of Casp3, Casp6, Bcl2, and Bax after normalization with Gapdh and Hprt1 between the PTU-alone group and untreated controls or between any antioxidant co-exposure group and the PTU-alone group.
On PND 77, no significant difference was observed in the transcript levels of glutamate receptor-related Gria1, Gria3, Grin2a, and Grin2b after normalization with Gapdh and Hprt1 in the PTU-alone group compared with the untreated controls (Table 2). The transcript levels of Gria1, Gria3, Grin2a, and Grin2b significantly increased after normalization with Gapdh and/or Hprt1 in the PTU + AGIQ group and PTU + ALA group compared with the PTU-alone group. No significant difference was observed in the transcript level of neurotrophic factor Bdnf after normalization with Gapdh and Hprt1 in the PTU-alone group compared with the untreated controls. The transcript level of Bdnf significantly increased after normalization with Gapdh in the PTU + AGIQ group and PTU + ALA group compared with the PTU-alone group. The transcript level of synaptic plasticity-related Arc significantly decreased after normalization with Gapdh and Hprt1 in the PTU-alone group compared with the untreated controls. No significant difference was observed in the transcript level of Efnb3 after normalization with Gapdh and Hprt1 in the PTU-alone group compared with the untreated controls. No significant difference was observed in the transcript levels of Arc and Efnb3 after normalization with Gapdh and Hprt1 between any antioxidant co-exposure group and the PTU-alone group. No significant differences were observed in the transcript levels of stem cell factor/receptor-related Kitlg and Kit after normalization with Gapdh and Hprt1 in the PTU-alone group compared with the untreated controls. Transcript level of Kitlg significantly increased after normalization with Gapdh in the PTU + ALA group compared with the PTU-alone group.
Lipid peroxidation level in the hippocampus of male offspring
On PND 21, there were no significantly different changes in MDA level in the hippocampus between the PTU-alone group and untreated controls or between any antioxidant co-exposure group and the PTU-alone group (Fig. 7).
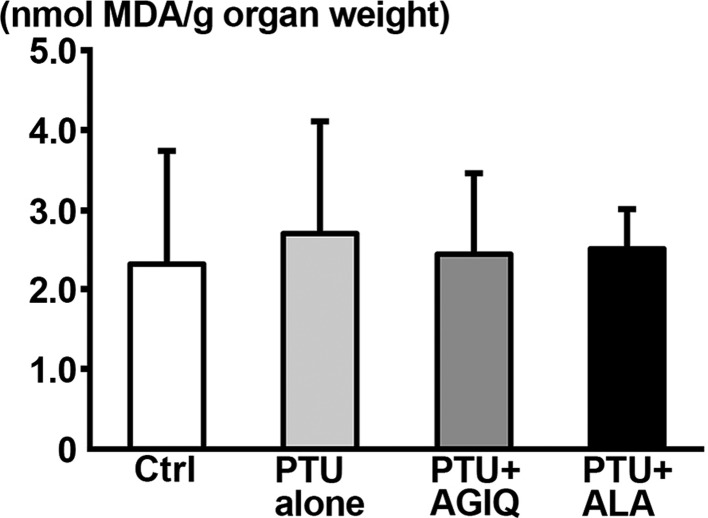
Fig. 7. Thiobarbituric acid reactive substances (TBARS) level of the dissected hippocampal tissue in male offspring on postnatal day (PND) 21 as measured by the amount of malondialdehyde (MDA). Data are expressed as the mean + SD. N=6–8 (untreated controls, 8; 6-propyl-2-thiouracil (PTU)-alone group, 6; PTU + α-glycosyl isoquercitrin (AGIQ) group, 7; PTU + α-lipoic acid (ALA) group, 7).
Discussion
We previously revealed that developmental hypothyroidism caused by maternal exposure to PTU at 10 ppm in drinking water decreased the number of GFAP+ cells, which represent type-1 stem cells, in the SGZ and the number of DCX+ cells, representing type-2b and type-3 progenitor cells and immature granule cells, in the SGZ and GCL in offspring at the end of exposure on PND 216. Consistent with the previous study, we observed a decreased number of GFAP+ cells and a tendency for a decrease in the number of DCX+ cells caused by PTU exposure at 12 ppm in the present study. We also observed decreases in the number of TBR2+ cells, which represent type-2b progenitor cells, in the SGZ and in the number of NeuN+ cells, which represent immature and mature granule cells, in the SGZ and GCL. The reason for the decreases in TBR2+ cells and NeuN+ cells caused by PTU exposure in the present study may be the larger PTU dose than the aforementioned previous study resulting in a stronger impact of hypothyroidism-related effects on neurogenesis. It is reported that thyroid hormone receptor (TR) α1 is expressed in type-2b progenitor cells and subsequent progenitor cells and postmitotic granule cells in the hippocampal granule cell lineages and that it controls survival and differentiation of these cellular subpopulations35. In the present study, the PTU dose of 12 ppm might have suppressed differentiation from TBR2+ cells to NeuN+ cells, in contrast to the suppression of only DCX+ cells at 10 ppm in our previous study6. On PND 77, we observed a sustained decrease of TBR2+ cells caused by 12-ppm PTU, in contrast to the recovery of most changes of granule cell lineage at 10 ppm in our previous study6, which suggested sustained disruption of neurogenesis caused by the large PTU dose.
Regarding GABAergic interneuron subpopulations, we previously revealed increased numbers of RELN+, CALB2+, and SST+ interneurons and a decreased number of PVALB+ interneurons in the DG hilar region on PND 21 caused by PTU at 10 ppm6. Consistent with the previous study, we observed a decrease in the number of the hilar PVALB+ interneurons in the PTU-alone group on PND 21. However, we did not observe changes in the number of RELN+, CALB2+, and SST+ interneurons. Although the cause of these differences is unclear, it may be possible that growth suppression of offspring caused by PTU exposure affects the neuronal distribution. In the present study, dams of the PTU-alone group only showed slight and transient decreases in body weight during exposure, while these dams revealed suppressed water consumption and food intake. Therefore, maternal undernutrition was unlikely to occur as a cause of progressive growth retardation of offspring in the present study. A stronger impact of retardation of body and brain growth as a secondary effect of PTU-induced hypothyroidism at 12-ppm than at 10-ppm probably influences the hypothyroidism-related molecular and cellular events in neural components during development. Body weight of male pups in the PTU-alone group on PND 21 in the present study was approximately 18% smaller than that of pups in 10 ppm group on PND 21 in our previous study6. In the present study, a decreased number of PVALB+ interneurons was sustained through PND 77 as previously reported36. Regarding synaptic plasticity-related cellular distribution changes in the GCL, we found a decreased number of ARC+ or FOS+ cells but unchanged number of COX2+ cells in the PTU-alone group on PND 21. We previously found decreased numbers of ARC+ cells and COX2+ cells but an unchanged number of FOS+ cells on PND 21 caused by developmental PTU exposure at 10 ppm6, 37. While the reason for the discrepancy in the numbers of FOS+ cells and COX2+ cells between the present and previous studies is not clear, speculation similar to that regarding a growth suppression effect as the cause of the different results for interneuron subpopulations could be made for the discrepancy in synaptic plasticity-related changes. On PND 77 in the present study, the number of ARC+ or FOS+ cells was reversed after cessation of PTU exposure.
One of the most important functions performed by thyroid hormones is the tight regulation of cellular oxygen consumption and consequent generation of ROS in several organs including the brain38, 39. However, there is a study reporting that PTU exposure in dams at 500 ppm in drinking water from birth to weaning on PND 25 and then in male offspring at the same concentration to PND 30 resulted in a decreased or unchanged lipid peroxidation level in the cerebral cortex on PNDs 7, 15, and 30 in rats40. While the dose level of PTU and brain region of interest are different from the present study, this result suggests that developmental hypothyroidism does not cause oxidative stress in the developing brain. In contrast, a slight increase of oxidative stress level has been reported in the developing rat hippocampus at the end of maternal exposure to 500 ppm PTU12. In the present study, we found that developmental hypothyroidism caused by PTU exposure at 12 ppm does not alter the lipid peroxidation level in the hippocampus at the end of exposure. The obtained results suggest that oxidative stress is not primarily involved in developmental hypothyroidism-related aberrations in hippocampal neurogenesis under our experimental conditions. PVALB+ GABAergic interneurons have TRs, and development and function of these interneurons are highly dependent on thyroid hormone41. Considering that PVALB+ interneurons play a role in maintaining neurogenesis3, 42, our present results may suggest that deprivation of thyroid hormone itself is primarily crucial for disruption of neurogenesis rather than induction of oxidative stress in the hippocampus during development.
In the present study, co-exposure of AGIQ or ALA with PTU did not prevent immunohistochemically revealed hypothyroidism-induced aberrant neurogenesis in the hippocampus. However, we observed restoration of the transcript levels of Eomes (also known as Tbr2), Gria3, Grin2a, Grin2b, and Epha4 in the DG on PND 21 caused by co-exposure of AGIQ or ALA with PTU in contrast to the decreased transcript level in the PTU-alone group as compared with the untreated controls. TBR2 is a transcription factor that is thought to be crucial for the progression of neuronal fate in the adult hippocampus1. Gria3 encodes one of the alpha-amino-3-hydroxy-5-methyl-4-isoxazole-propionic acid receptor (AMPAR) subunits of glutamate receptors43. AMPAR is widely distributed in the brain and can control synaptic plasticity via influx of Ca2+ into neurons44. Grin2a and Grin2b encode one of the N-methyl-d-aspartate receptor (NMDAR) subunits of glutamate receptors, i.e., GluN2A and GluN2B. Both subunits are involved in regulation of bidirectional synaptic plasticity, i.e., long-term potentiation and long-term depression in the hippocampus45. NMDAR activation regulates adult neurogenesis of the hippocampus46. Epha4 encodes one of the EPH receptors and has been implicated in the regulation of dendritic spine morphology and neurogenesis in the hippocampus47, 48. Therefore, restoration of the transcript levels of these genes by AGIQ or ALA co-exposure suggests enhancement of synaptic plasticity and neurogenesis in the hippocampus by antioxidant co-exposure. In agreement with these changes, we found increased transcript levels of Gria1, Gria2, Bdnf, Arc, Efnb3, and Ptgs2 in the DG caused by antioxidant co-exposure as compared with PTU alone on PND 21, and all of these genes have been shown to play roles in synaptic plasticity35, 44, 49, 50, 51. Moreover, we found increased transcript levels of Dcx, Reln, Sst, Kit, Igf1r, and Igf2r and downregulation of Kitlg in the DG on PND 21 caused by co-exposure of AGIQ or ALA with PTU as compared with PTU alone. DCX is known to be associated with migration of these neuroblasts52. RELN is expressed in inhibitory interneurons and is thought to be involved in regulation of neuronal precursor cell migration53. Therefore, upregulation of DCX and RELN may be associated with facilitation of neurogenesis involving neuronal precursor cell migration. With regard to SST, this protein is expressed in GABAergic inhibitory interneurons that play a vital role in neural circuitry and activity54. It is also reported that BDNF upregulates Sst at the transcription level as revealed in a transfection study using rat primary cultured cerebrocortical neurons55. BDNF is synthesized by hippocampal granule cells and has neuronal growth effects on hilar interneurons through TRKB receptor activation and promotes differentiation and maturation of progenitor cells in the SGZ56. As mentioned above, we observed transcript upregulation of Bdnf caused by antioxidant co-exposure on PND 21 in the present study. These results suggest that an increase of SST+ interneurons may be caused by BDNF-TRKB signaling to reflect a compensatory response for progenitor cell proliferation against reduced type-1 stem cells. Kit encodes stem cell growth factor receptor KIT, and signals of KIT and SCF encoded by Kitlg stimulate neurogenesis in vitro and in vivo57. In the present study, Kit upregulation caused by antioxidant treatment may reflect progenitor cell proliferation. However, the downregulation of Kitlg in these animals suggested suppression of SGZ cell proliferation in these animals. Igf1r and Igf2r, encoding insulin-like growth factor receptor IGF1R and IGF2R, also regulate adult neurogenesis of the hippocampus58, 59. Therefore, upregulation of both receptor genes suggested an increase of the sensitivity for IGF-mediated proliferation signals of SGZ cells.
As already mentioned, we did not find any alteration in the hippocampal lipid peroxidation level at the end of developmental PTU exposure. Moreover, co-exposure of AGIQ or ALA with PTU also did not alter the lipid peroxidation level. These results suggest that some unknown mechanism different from an antioxidant effect against oxidative stress responses was operated for the restorative effect of AGIQ and ALA on transcript expression changes of many genes at the end of developmental hypothyroidism. It is now understood that the biological actions of naturally occurring antioxidants, such as flavonoids, within the nervous system are not due to their direct (i.e., classical) antioxidant effects60 but rather are due to indirect effects through their potential to protect vulnerable neurons, enhance existing neuronal function, stimulate neuronal regeneration, and induce neurogenesis60, 61.
In the present study, despite the transcript expression changes suggestive of enhancement of synaptic plasticity and neurogenesis, immunohistochemical results did not support these changes. On the other hand, thyroid hormone has been shown to be involved in the stabilization of mRNA and facilitation of translation through cytoskeletal rearrangement62. Therefore, increased transcripts by co-administration of AGIQ or ALA may not effectively be translated into peptides due to hypothyroidism in the present study. On PND 77, higher transcript levels were observed in Gria1, Gria3, Grin2a, Grin2b, Bdnf, and Kitlg in the DG caused by co-exposure of AGIQ or ALA with PTU as compared with PTU alone. Increased expression of these transcripts may reflect operation of a compensatory mechanism for sustained reduction of TBR2+ cells and PVALB+ cells at the adult stage.
In conclusion, the results of the present study revealed that disruption of hippocampal neurogenesis induced by hypothyroidism in the developmental stage was not prevented by co-exposure to AGIQ or ALA. Developmental hypothyroidism and additional treatment with an antioxidant did not alter the hippocampal lipid peroxidation level. The obtained results suggest a possibility that oxidative stress is not primarily involved in the disruption of neurogenesis during development induced by hypothyroidism. Gene transcript expression changes across multiple functions caused by co-exposure of an antioxidant at the end of developmental hypothyroidism may be the result of neuroprotective actions of antioxidants rather than antioxidant activity. However, no preventive effect on neurogenesis in the present study suggested impairment of protein synthesis via an effect on mRNA stability and translation due to hypothyroidism.
Disclosure of Potential Conflicts of Interest
Mihoko Koyanagi and Shim-mo Hayashi are employed by a food additive manufacturer whose product lines include α-glycosyl isoquercitrin. The views and opinions expressed in this article are those of the authors and not necessarily those of their respective employers. Yasunori Masubuchi, Takaharu Tanaka, Rena Okada, Yuko Ito, Junta Nakahara, Satomi Kikuchi, Yousuke Watanabe, Toshinori Yoshida, Robert R. Maronpot, and Makoto Shibutani declare that no conflicts of interest exist.
Acknowledgments
The authors thank Mrs. Shigeko Suzuki for her technical assistance in preparing the histological specimens. This work was supported by San-Ei Gen F.F.I., Inc.
References